|
Galaxea fascicularis (Linnaeus 1767)
|
|
Courtney Brooke Davies 2017
|
|
|
Summary | |
Galaxea fascicularis, commonly known as galaxy coral, starburst coral or fluorescence grass coral to name a few, is a type of stony coral belonging to the family Oculinidae. Their name relates to the polyp's superficial resemblance to a star when the tentacles are fully extended, which occurs during the daytime unlike some corals whose polyps remain retracted until dark when active feeding occurs. As one name also suggests, this coral, like many Cnidarians, has specific proteins called green fluorescent proteins that enable it to fluoresce a brightly coloured green when exposed to specific wavelengths of light. The abundant photosynthetic, dinoflagellate algae known as zooxanthellae that inhabit the tissue of G. fascicularis also fluoresce due to their red fluorescent protein and are an integral part of the coral's physiology.
Newly settled polyps, also known as coral spat, were identified on settlement plates that were collected from Manly Boat Harbour in Moreton Bay, thus no fully grown coral specimen is available for investigation. In contrast however, the progression of polyps through very small, relatively newly settled spats to approximately four to five months old could be observed and photo documented, which has seldom been done before. A more in depth investigation into the density of zooxanthellae within the polyp and the physiological implications of this was explored further and details of this and many other aspects of this interesting specimen shall be examined throughout this web page.
|
|
|
Physical Description |
Identifying Characters | |
G. fascicularis is a hermatypic coral that grows in various forms depending on the size of the colony; low domes, cushion-shapes, or irregular growths are typical of small colonies, while large colonies exhibit columnar or massive growth forms and can grow in excess of five metres across (Crabbe & Smith 2006; Veron & Pichon 1979). Individual corallites typically vary in size reaching six to ten millimetres with the centre being a point of intersection for a number of exsert septa, thus columellae are either very fragile or not present. The septa can also be felt through the tissue of the polyp and observed in live specimens when the polyp retracts into the calyx (Figure 1), however during the day tentacles tend to be extended. Another defining characteristic of the corallites based on the species' inclusion in the genus Galaxea is that they are thin-walled and cylindrical with a blister-like coenosteum separating them.
Figure 1. Two G. fascicularis polyps showing the difference between a polyp retracted into the calyx revealing septa (left) and a normal polyp with tissue fully covering the septa (right).
|
|
|
Morphology | |
As happens with most corals, a colony starts out when the larvae settle on the substrate and begin to secrete a skeleton of calcium carbonate as can be seen surrounding the polyps in Figure 2 (Al-Horani et al. 2005). The polyp continues to secrete more layers of skeleton over the basal plate, lifting it up, and directly underneath the tissue of the polyp a cup-like calyx is secreted in which the polyp resides (Al-Horani et al. 2005). The skeleton of the polyp is termed the corallite and the skeleton between corallites is the coenosteum while the living tissue over this is the coenosarc (Al-Horani et al. 2005). As seen in Figure 2, this clump of polyps share a skeleton and thus coenosarc, indicating that they may be genetically identical clones that were formed through budding from the first individual polyp that settled. However, it may also be possible that they were individual polyps that have joined together, if their genetic material were similar enough to allow for this to happen.
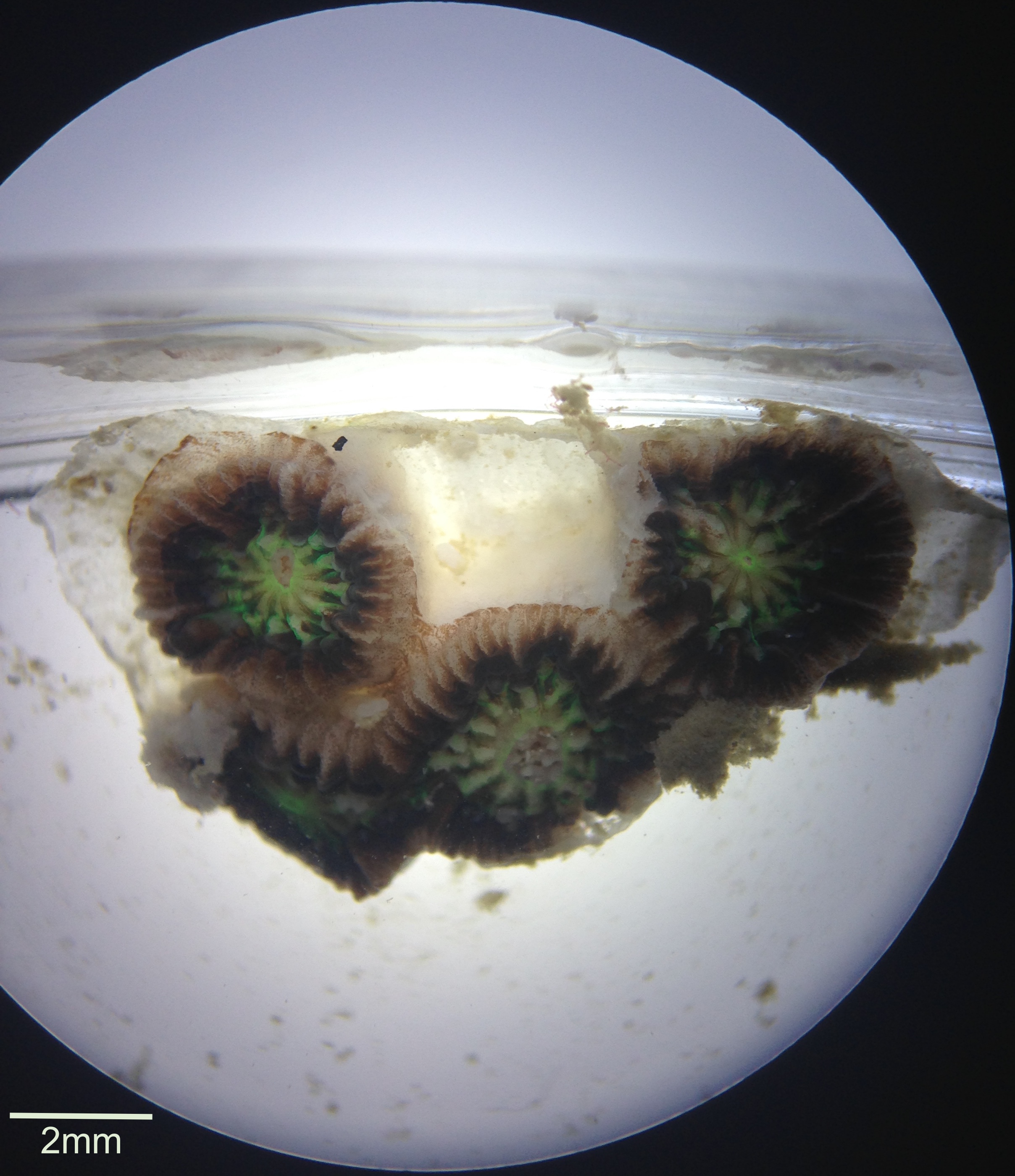
Figure 2. The calcium carbonate skeleton secreted by and surrounding the four polyps can be clearly seen in this image.
In Figure 3a the same individual as Figure 1 is displayed retracted into the calyx, however one week later it was observed expanding its body and tentacles but not over the whole area of its skeleton like normal, which can be seen in Figure 3b. This may be indicative of it potentially resorbing its skeleton, possibly as a result of stressful or suboptimal conditions. While classified in general as a stony (scleractinian) coral, G. fascicularis colonies can also be differentiated into either soft or hard types based on morphology of their nematocysts; soft colonies are those with long shafted and wide capsuled nematocysts while nematocysts with short shafts and thin capsules are representative of hard colonies (Nakajima et al. 2015). Colonies with a mixture of these two forms can also be found.
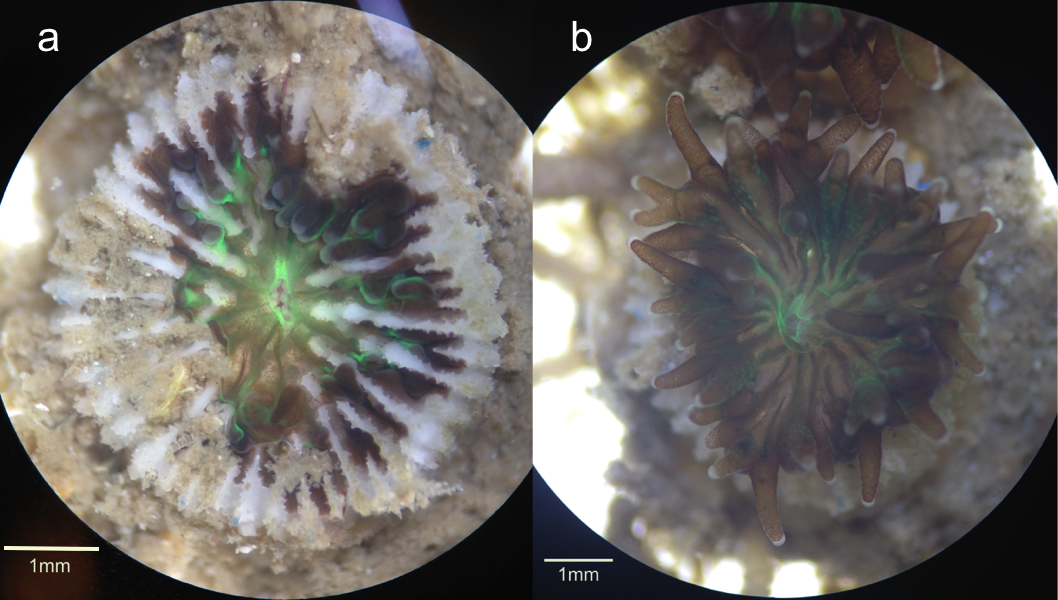
Figure 3. (a) Polyp retracted into calyx revealing septa. (b) The same polyp with tentacles extended, however the tissue is not expanded over the whole skeleton thus septa are still visible around the perimeter underneath the tentacles.
G. fascicularis exhibits a range of morphotypes that can vary depending on a range of variables. Crabbe & Smith (2006) have shown that depth and incident light cause significant changes in various features of corallites of G. fascicularis such as height, width, density, and distance between corallites. It was found that with increasing incident light there was a decrease in corallite width, increase in corallite height, and decrease in inter-corallite distance. These conditions relate to colonies where light conditions are favourable such as on the reef crest or upper reef slope, and where the arrangement of corallites is in such a way that the surface area for photosynthesis is maximised as there is potential for more zooxanthellae to be present (Crabbe & Smith 2006). In contrast, in colonies found further down the reef slope or in turbid waters where light is a limiting factor, heterotrophy is favoured and thus larger corallites that are further apart may have more tentacles that they can also extend further to increase the capture of food (Crabbe & Smith 2006).
Many colour variations exist in this species with colours often contrasting between the tentacles and oral disc or between the polyp and the septa (Veron 2000). The main colour morphs identified include: polyps that are pale brown, polyps brown with a green oral disc, polyps brown with tentacles of the major septa green, polyps brown with green external tentacles, polyps brown with tentacles of a pale green (almost white) flourescent colour, and polyps brown with green fluorescent tentacles (Abe et al. 2008; Hidaka & Yamazato 1985). This specific specimen is of the brown with green oral disc colour morph. Having a conspicuous white tip at the end of the tentacle also identifies the tentacles of G. fascicularis that tend to be 3-5mm in length (Hidaka & Yamazoto 1984). Interestingly, some polyps were observed to have bifurcate tentacles as seen in Figure 4. This has not been documented in the literature and is assumed to be an unusual abnormality. The central disk contains a single siphonoglyph from which a varying number of tentacles extend that are classified as either being septal (extend from septa and are the ventral most tentacles) or external (sit underneath the septal tentacles) (Abe et al. 2008). Zooxanthellae are visibly present on the tentacles and oral disc as depicted in Figure 5, but are also considerably abundant further within the coelenteron (Al-Horani et al. 2005).
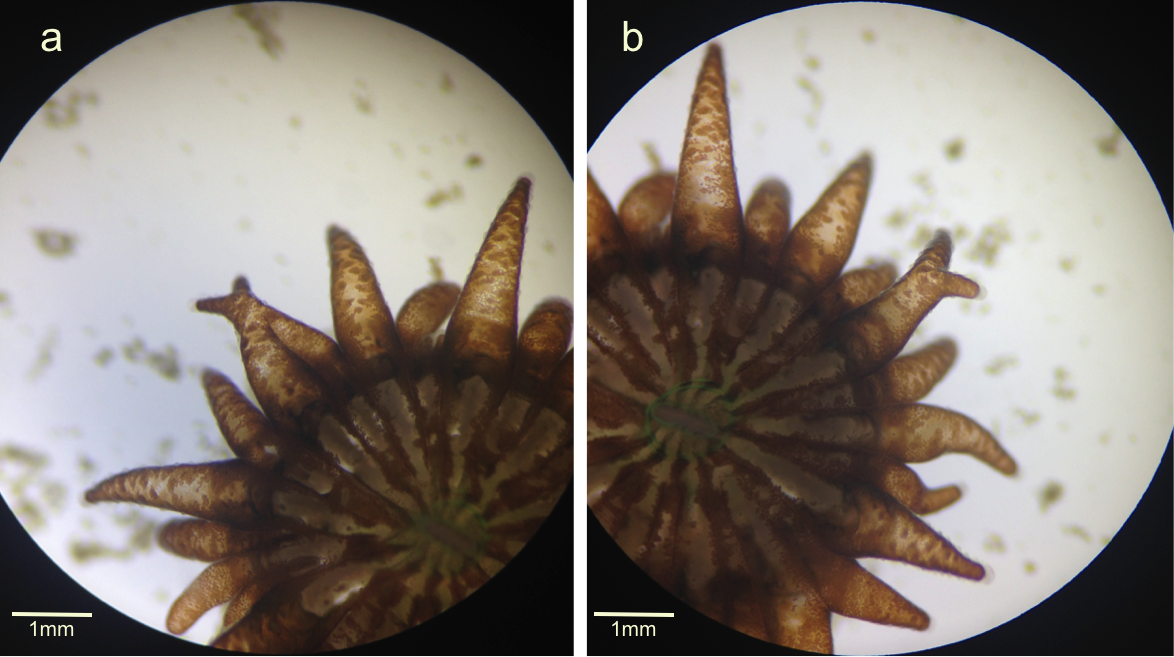
Figure 4. (a) The tentacle in the centre of the picture appeared to begin as two separate tentacles that then joined together before separating again at the tip. (b) The same individual with the tentacle in the centre of the picture only splitting at the tip this time.
Figure 5. A quarter of an individual polyp with zooxanthellae (brown dots) clearly visible in the tissue over the whole polyp body.
|
|
|
|
Ecology |
Habitat | |
G. fascicularis can be found in a wide range of habitats owing to their high resilience to stress from bleaching and from sedimentation given their ability to rapidly remove sediment via ciliary action (Crabbe & Smith 2005; Junjie et al. 2014). They are a dominant species of inshore fringing reefs where they tend to prefer sheltered areas that do not receive a lot of strong wave action and may inhabit the reef crest, slope or deeper parts of the reef flat ranging from depths of three to 25 metres (Veron 2000; Crabbe & Smith 2005; Veron & Pichon 1979). Many large colonies tend to occur in habitats with lower levels of light, in particular water that is turbid and subject to tidal currents (Veron & Pichon 1979).
It should be noted that the specimens of G. fascicularis spat found in the laboratory were attached to settlement plates that had been collected from Manly Boat Harbour. Given that coral larvae require a specific set of settlement cues in order for settlement to be induced, then it may be interesting to investigate this, as a settlement plate surely contains considerably different cues to those given off by a suitable location on a fringing reef. This may be indicative of a biofouling existence, which is plausible given the dominant nature of G. fascicularis in reef ecosystems.
|
|
|
Symbioses | |
Symbiotic dinoflagellates, Symbiodinium sp., are an integral part of the endodermal cells of hermatypic corals, with G. fascicularis relying mainly on the organic material produced by the algae’s photosynthesis to support most of their energetic requirements (Kunjie et al. 2014). Given this fact, and the high densities of zooxanthellae observed in specimens through a dissecting microscope, it was wondered whether the densities or distribution of zooxanthellae in the specimen’s tentacles changed as the polyp grew larger. As seen in Figure 6 of a dissected tentacle from a fixed specimen classified as being small in size (settled relatively recently), zooxanthellae occupy virtually all endodermal tissue. This was the same for the fixed specimen’s tentacles that came from a large polyp. Interestingly, tentacles from a polyp of intermediate size had a break in zooxanthellae just before the tip (Figure 7). The reason for this is not clear and it may be a morphological variation not related to size but rather different water conditions, as this specimen was fixed a week earlier than the others and came straight off the settlement plate, while the others were kept in a small plastic container in the university’s aquarium for a week before being fixed. Figure 8 depicting a planula coral larva that was found in association with the polyps but may not necessarily be of the same species was also found to have extremely high densities of zooxanthellae throughout the whole body.
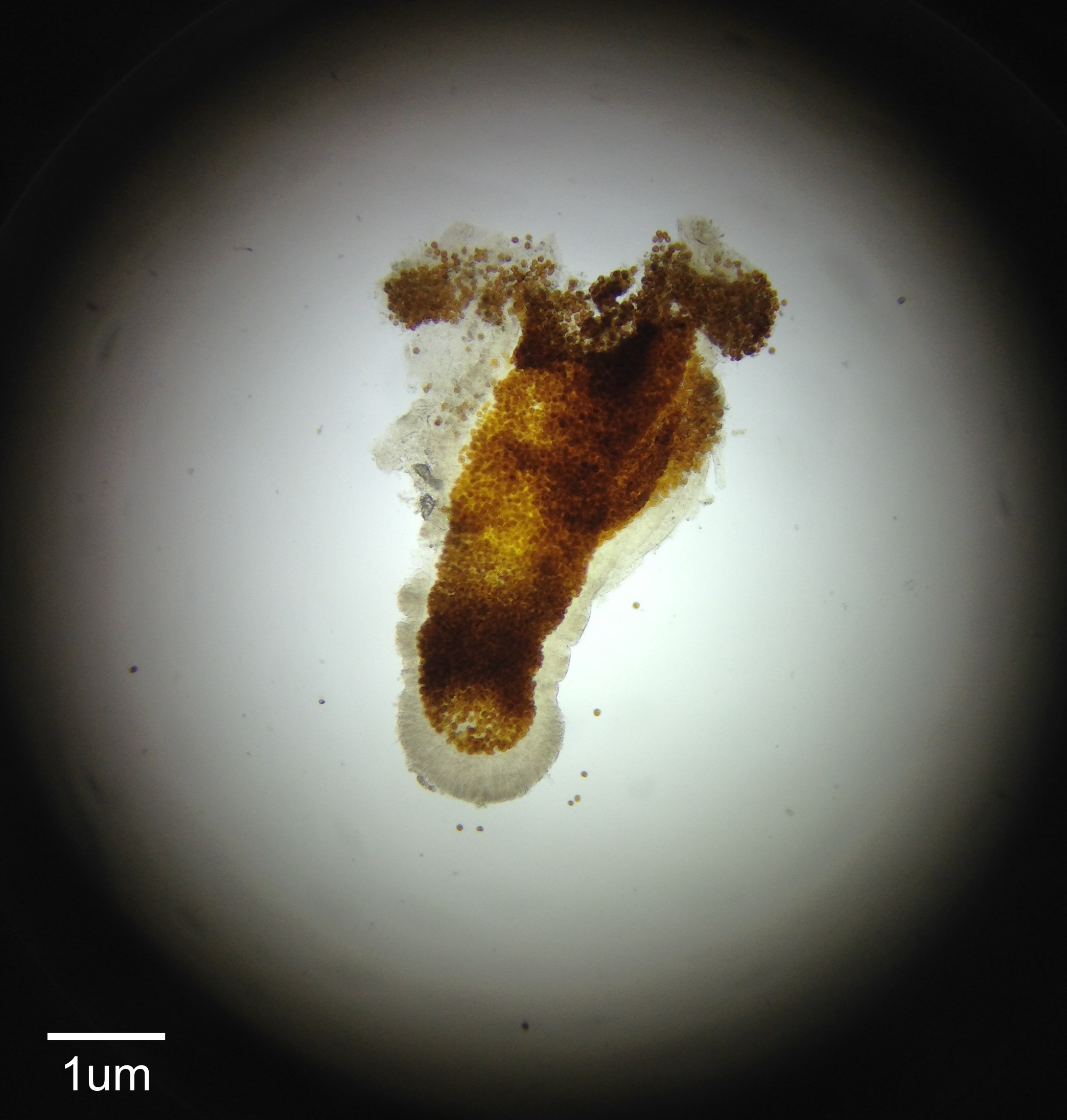
Figure 6. A dissected tentacle from a fixed specimen that was small in size viewed under 10x magnification. Abundant zooxanthellae visible as brown dots throughout the whole tentacle.
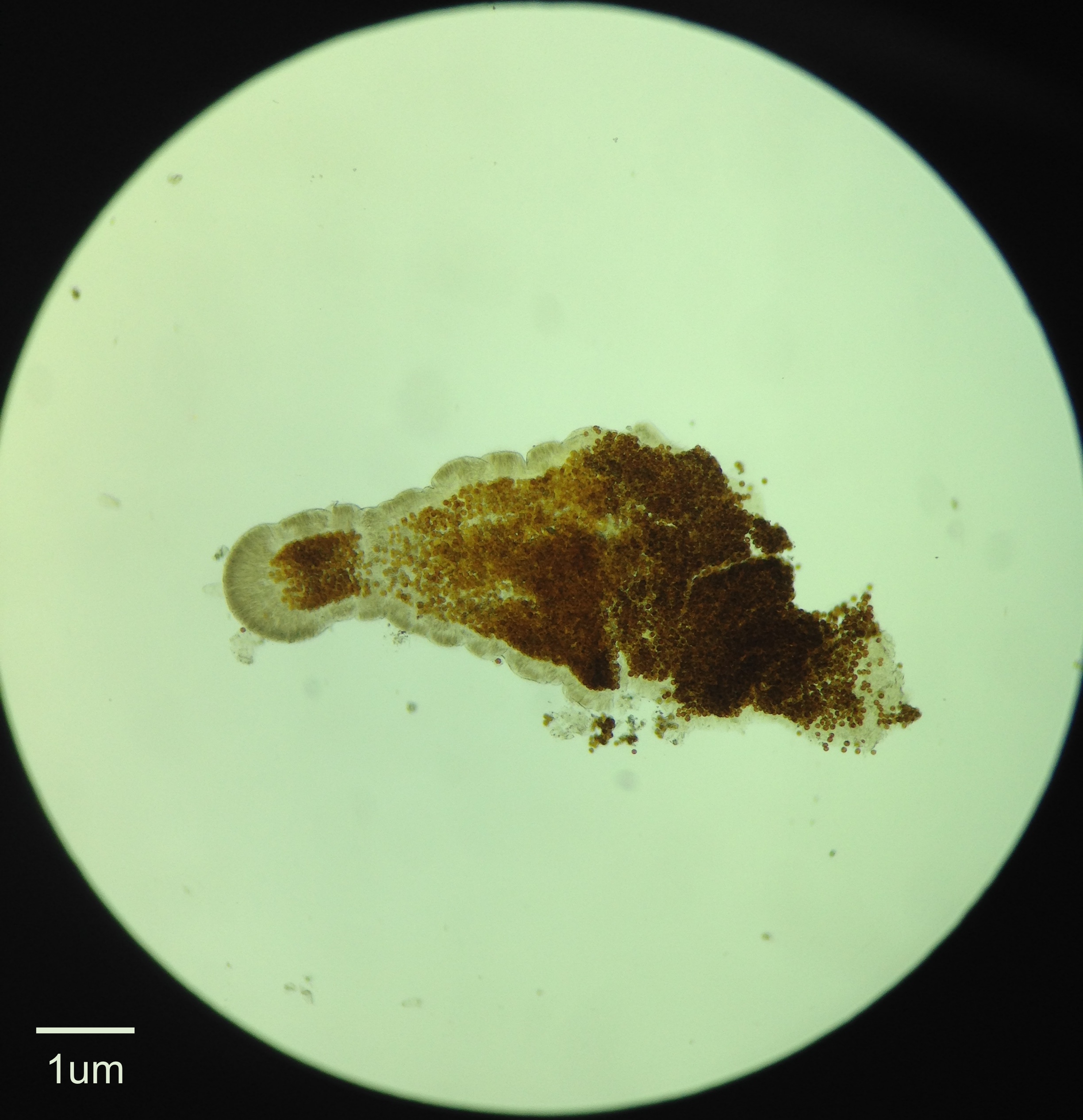
Figure 7. A dissected tentacle from a fixed specimen that was medium in size viewed under 10x magnification. A section of reduced zooxanthellae is visible just before the tip of the tentacle.
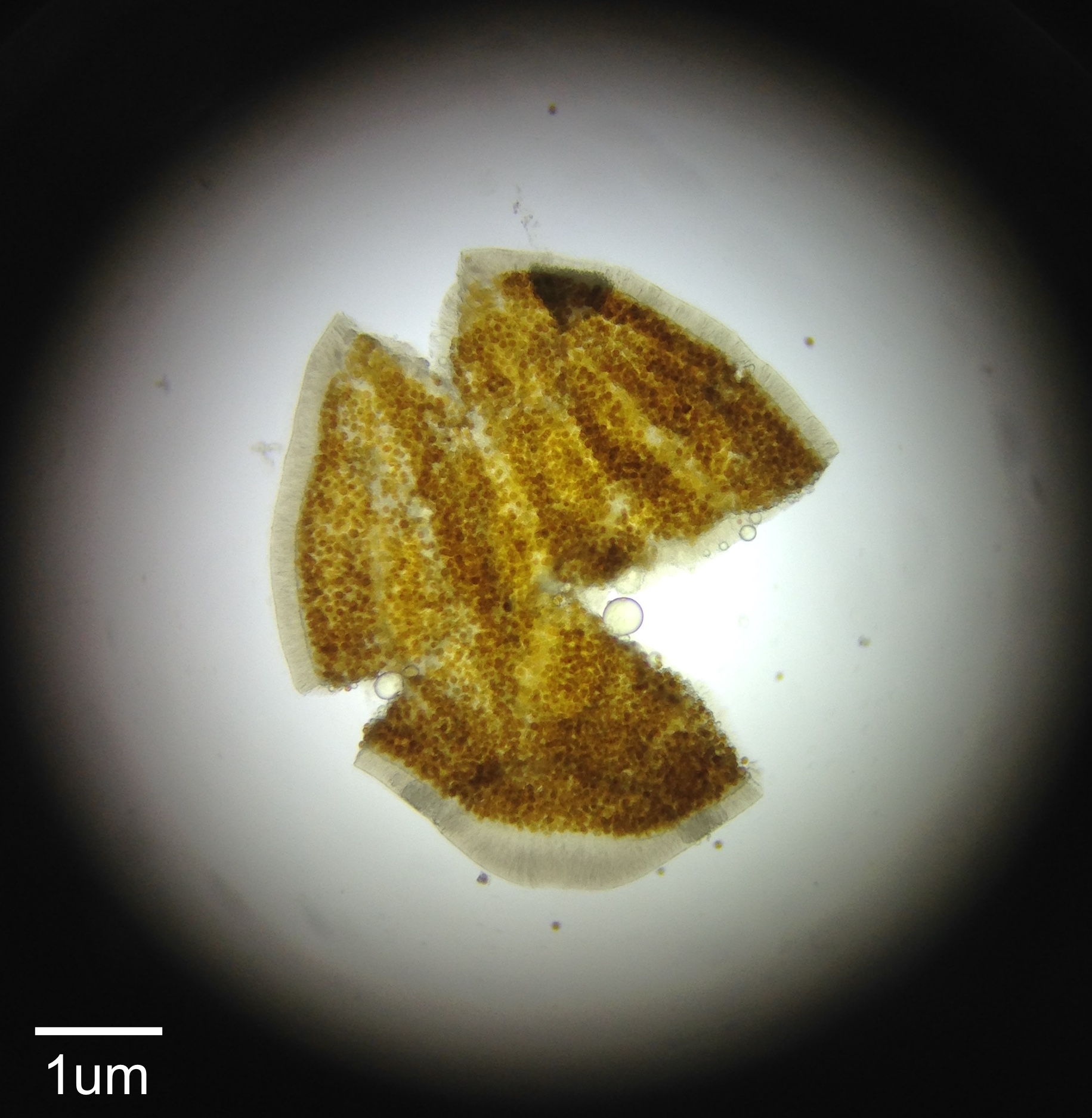
Figure 8. Fixed specimen of a coral planula larvae viewed under 10x magnification with abundant zooxanthellae visible throughout.
Different clades of Symbiodinium sp. tend to be species specific with many corals being inhabited by only one clade. G. fascicularis colonies however, have been found to be host to both Symbiodinium clade C and clade D (Dong et al. 2009; Zhou et al. 2011). Huang et al. (2011) also found that latitudinal variation exists within this species where colonies from high latitudes in Japan contained only Symbiodinium clade C while lower latitude colonies were host to either Symbiodinium clade C or D sequentially or simultaneously. These trends are repeated in studies undertaken on G. fascicularis colonies from various locations within their distribution, with Symbiodinium clade D appearing to be confined to colonies of lower latitudes (Chen et al. 2005; Visram & Douglas 2006; LaJeunesse et al. 2004; McClanahan et al. 2005; Huang et al. 2011). The fact that G. fascicularis has the flexibility to be associated with two clades of Symbiodinium is ecologically important as these zooxanthellae taxa may confer an advantage against bleaching, especially clade D, whereby the ability to adapt to changing environmental conditions is enhanced and thus survival against mass coral bleaching may also be increased (Huang et al. 2011). This is of significant importance during this time of climate change where many species of coral are becoming increasingly bleached and there is fear of these populations not recovering. It may also be possible then, that the extremely high densities of zooxanthellae observed in the tentacles correlates to this advantage, with colonies containing higher densities possibly benefitting even more so than those with lower densities. Further research is needed to determine whether this may be the case.
As well as symbiotic algae, many coral species tend to have symbiotic relationships with other marine invertebrates, in particular epizoic acoelomorph flatworms. In the case of G. fascicularis, polyps have been found to be the host of flatworms from the genus Waminoa (Wijgerde et al. 2013). This relationship has been found to negatively affect the coral in a number of ways. For example, light-shading occurs when the presence of flatworms on coral polyps and coenenchyme limit the amount of light the coral’s zooxanthellae can utilise, thus hindering the holobiont’s productivity (Barneah et al. 2007). The coral’s ability to feed on zooplankton is also inhibited by the flatworms and can occur in four possible ways (Wijgerde et al. 2013). The first way involves the flatworm directly competing for zooplankton prey with the host by capturing this prey before the coral as it comes into close proximity (Wijgerde et al. 2013). The second way is by physically obstructing the coral polyp’s oral disc, which results in fewer tentacles responding to food stimuli thus decreasing feeding efficiency (Wijgerde et al. 2013). Another mechanism involves the flatworm removing mucus from the oral disc that hinders the polyp’s ability to capture prey (Wijgerde et al. 2013). This also potentially reduces the resistance of the coral to pathogens and environmental changes (Barneah et al. 2007). Lastly, kleptoparasitism directly decreases the rate at which the coral can retain prey as the flatworm directly steals it from the coral (Wijgerde et al. 2013).
|
|
|
|
Life History and Behaviour |
Reproduction | |
Like all corals, G. fascicularis can reproduce both asexually and sexually. Asexual reproduction occurs in numerous forms and results in genetically identical individuals. Most commonly, asexual reproduction occurs by budding, where a new individual forms off the side of another, or fragmentation when a broken off part of the colony forms a new colony (Scott 1984). The primary type of budding exhibited by G. fascicularis is an identifying feature of the genus Galaxea and is known as extratentacular budding, which occurs at the colony margin where new corallites are formed outside of the corallite wall (Veron & Pichon 1979). It has also been observed in an aquarium setting however, that G. fascicularis colonies underwent intratentacular budding, whereby the corallite splits into two to form the new corallite and this occurs within the corallite wall (Nakano 2009).
The sexual reproductive mode of G. fascicularis is unique in that it is considered pseudo-gynodioecious, whereby colonies of this species can either be female or hermaphroditic (Harrison 1989; Keshavmurthy et al. 2012). Classified as broadcast spawners, mass spawning occurs twice a year after the full moon where female colonies release pink eggs, while hermaphroditic colonies produce white eggs that are very lipid-dense, as well as sperm (Keshavmurthy et al. 2012; Nakajima et al. 2016). While the pink eggs of female colonies are capable of being fertilized by the hermaphroditic sperm to produce planula larvae, the white eggs tend to remain unfertilized due to their large spheres of lipids inside them making it difficult for sperm to penetrate them (Keshavmurthy et al. 2012; Harrison 1989). Instead, these eggs appear to function in increasing the success rates of fertilization as they aid in lifting the bundles of sperm up to the water surface where the pink eggs float (Keshavmurthy et al. 2012). New research suggests that G. fascicularis may actually be gynodioecious however, as white eggs examined in this study were fertile and capable of undergoing embryogenesis in vivo (Keshavmurthy et al. 2012). This difference between the abilities of white eggs to be fertilized may simply be due to geographical variation between species. Nevertheless, this mode of reproduction results in a typical biphasic life cycle via production of a planktonic planula larvae and benthic adult coral colonies (Scott 1984; Rieger 1994).
|
|
|
Development | |
G. fascicularis undergoes what is known as complex development and results in the development of free swimming planula larvae (Okubo et al. 2013). After fertilization has occurred, the embryos firstly undergo holoblastic cleavage (Okubo et al. 2013). Gastrulation then occurs as the embryo progresses through a compressed stage of two layers before becoming spherical with smoothening of the cell surface, thickening of the blastula by elongation at right angles of cells to the flattened disc and formation of the blastopore through invagination of the sides of the embryo (Okubo et al. 2013). This leads to larvae spherical in shape with cilia allowing for rotary swimming approximately 18 hours after fertilization (Okubo et al. 2013). 48 hours post-fertilization sees some larvae become elongated, with high mobility being observed on the third day, and larvae forming mesenteries and becoming pear-shaped by the fourth day (Babcock & Heyward 1986). G. fascicularis larvae may be lecithotrophic or planktotrophic but most tend to become competent and settle and metamorphose within four and a half to five days post-fertilization when settlement cues are exactly right (Scott 1984; Babcock & Heyward 1986). This is the dispersal stage of the life cycle that enables the large distribution of the coral in widespread geographic regions and also results in essential population connectivity (Scott 1984; Nakajima et al. 2016). After settlement the larvae becomes attached to the substrate and begins to grow into a polyp with out-pockets near the mouth producing tentacles and a calcium carbonate skeleton secreted at the base (Ruppert, Fox & Barnes 2004).
|
|
|
Feeding | |
Corals are polytrophic, meaning they have the ability to acquire their nutritional requirements through either heterotrophic means or via their symbiotic zooxanthellae (Hii, Soo & Liew 2008). Heterotrophy is a vital part of the coral’s acquisition of nutrients as it supplies essential elements such as carbon, phosphorous and nitrogen to the coral and its zooxanthellae (Wijgerde et al. 2011). This is especially important during bleaching events where the corals are without zooxanthellae and must rely fully on heterotrophic feeding to obtain the nutrients they require (Grottoli, Rodrigues & Palardy 2006). The coral’s carnivorous process of feeding occurs by the polyp extending its tentacles into the surrounding environment in order to catch zooplankton, often producing mucus on the tentacles to increase capture efficiency (Hii, Soo & Liew 2008). When prey hit the tentacles, cnidocytes are also released to stun and ensnare the prey (Brusca, Moore & Shusca 2016). Mucociliary action then aids in moving the captured food to the oral disc where ingestion occurs (Wijgerde et al. 2013; Wijgerde et al. 2011). Food can either be digested internally or extracoelenterically (outside the coelenteron) by mesenterial filaments extending through the mouth of the polyp (Smith et al. 2016). In Figure 9 these filaments can be seen through the polyp’s mouth. The majority of G. fascicularis’s energy needs however, are supplied through the latter process by the photosynthetic production of organic compounds by their zooxanthellae (Junjie et al. 2014).
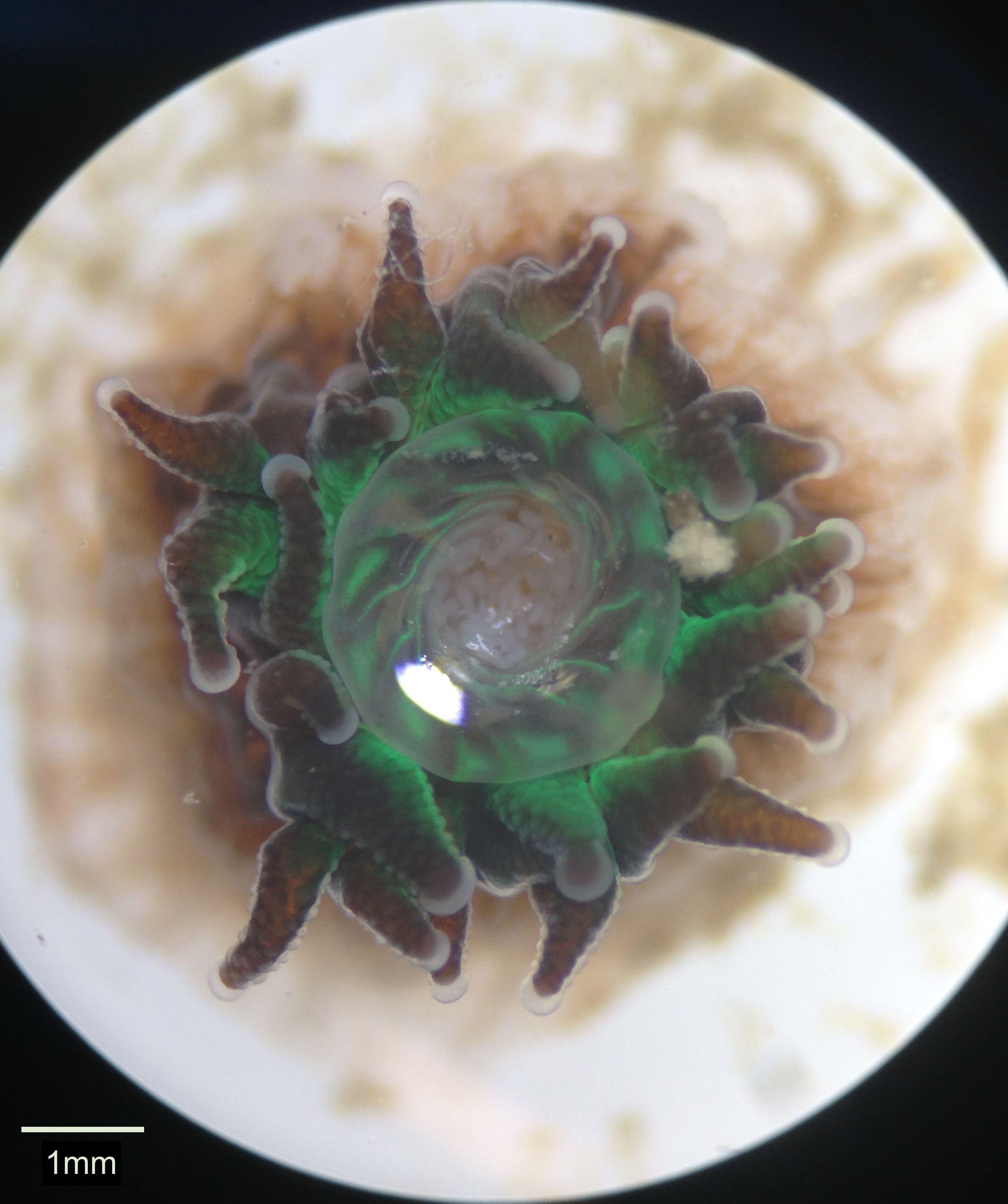
Figure 9. A live polyp with its mouth open displaying white mesenterial filaments located within the coelenteron.
|
|
|
|
Anatomy and Physiology |
General Anatomy | |
Coral colonies are made up of a network of polyps that are typical of the class Anthozoa as depicted in Figure 10. The colonial form of G. fascicularis is classified as coenosarcal as each polyp is interconnected via the coenosarc (Brusca, Moore & Shusca 2016). With a body plan of radial symmetry and an oral-aboral axis, the body wall of the polyp consists of two germ layers that form during embryogenesis – the epidermis and gastrodermis – and a third middle mesoglea layer that is derived from the ectoderm in adults (Brusca, Moore & Shusca 2016). Beneath the epidermis is the coelenteron (gastrovascular cavity) lined by the gastrodermis, between which the mesoglea resides (Brusca, Moore & Shusca 2016). The coelenteron has a high degree of compartmentalisation by mesenteries in anthozoan polyps that extend from the body wall, which have a gastrodermal lining and mesenchyme within them, and can be complete or incomplete depending on whether they fuse with the pharynx or not (Brusca, Moore & Shusca 2016). Mesenterial filaments (depicted in Figure 11) have a thickened edge below the pharynx that contains cnidae, cilia and gland cells that aid in their use for defence and the capture of prey (Brusca, Moore & Shusca 2016). The mouth occurs in the centre of the oral disc and leads to a muscular pharynx from which the coelenteron extends (Brusca, Moore & Shusca 2016). A variable number of tentacles radiate from the oral disc of G. fascicularis as one of three kinds; most commonly seen are septal and lateral tentacles, however sweeper tentacles may also be present, which are much longer than the others and have a defensive function (Hidaka & Yamazoto 1984).
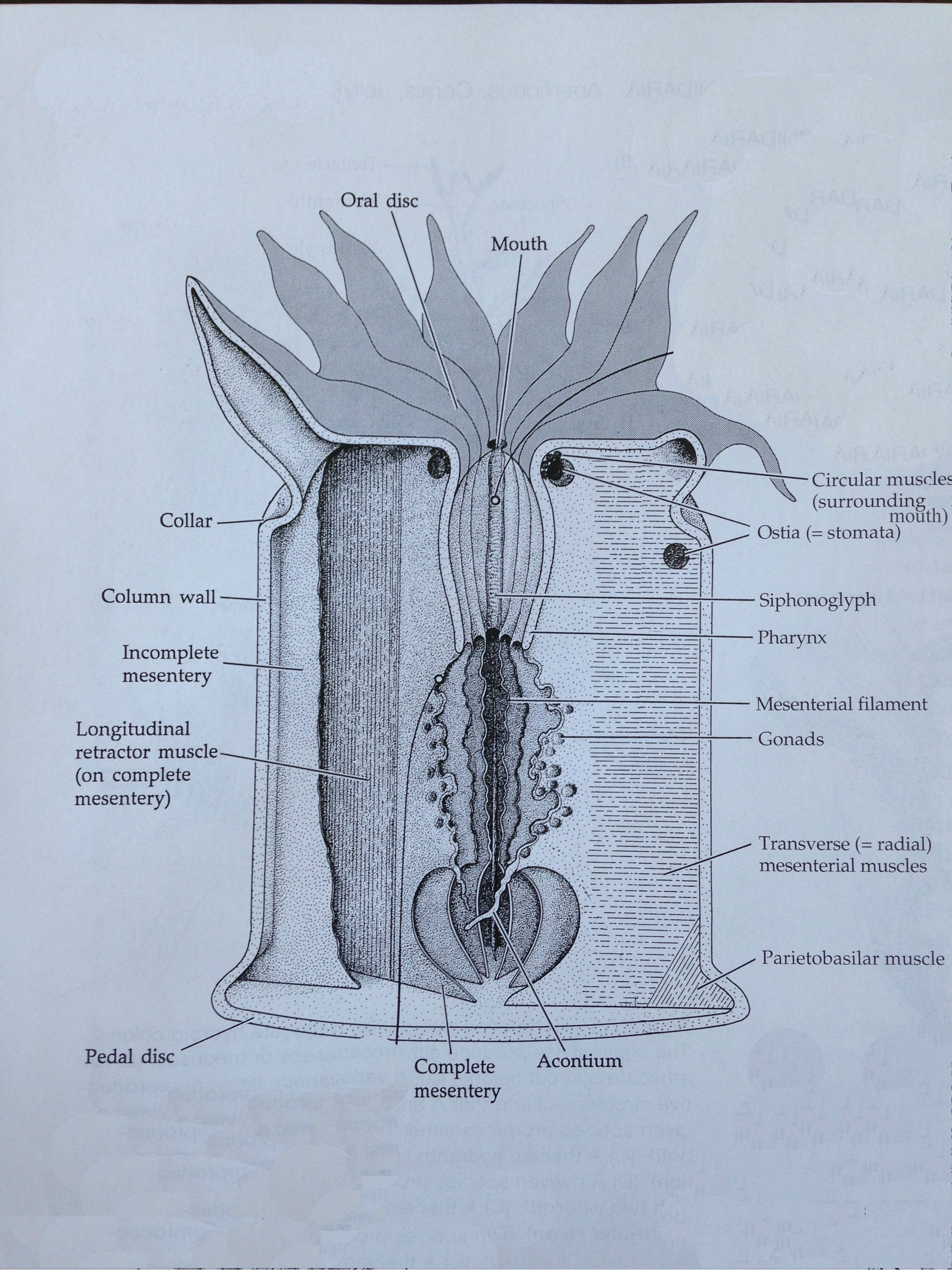
Figure 10. The basic internal anatomy of an Anthozoan polyp (adapted from Brusca, Moore & Shusca 2016).
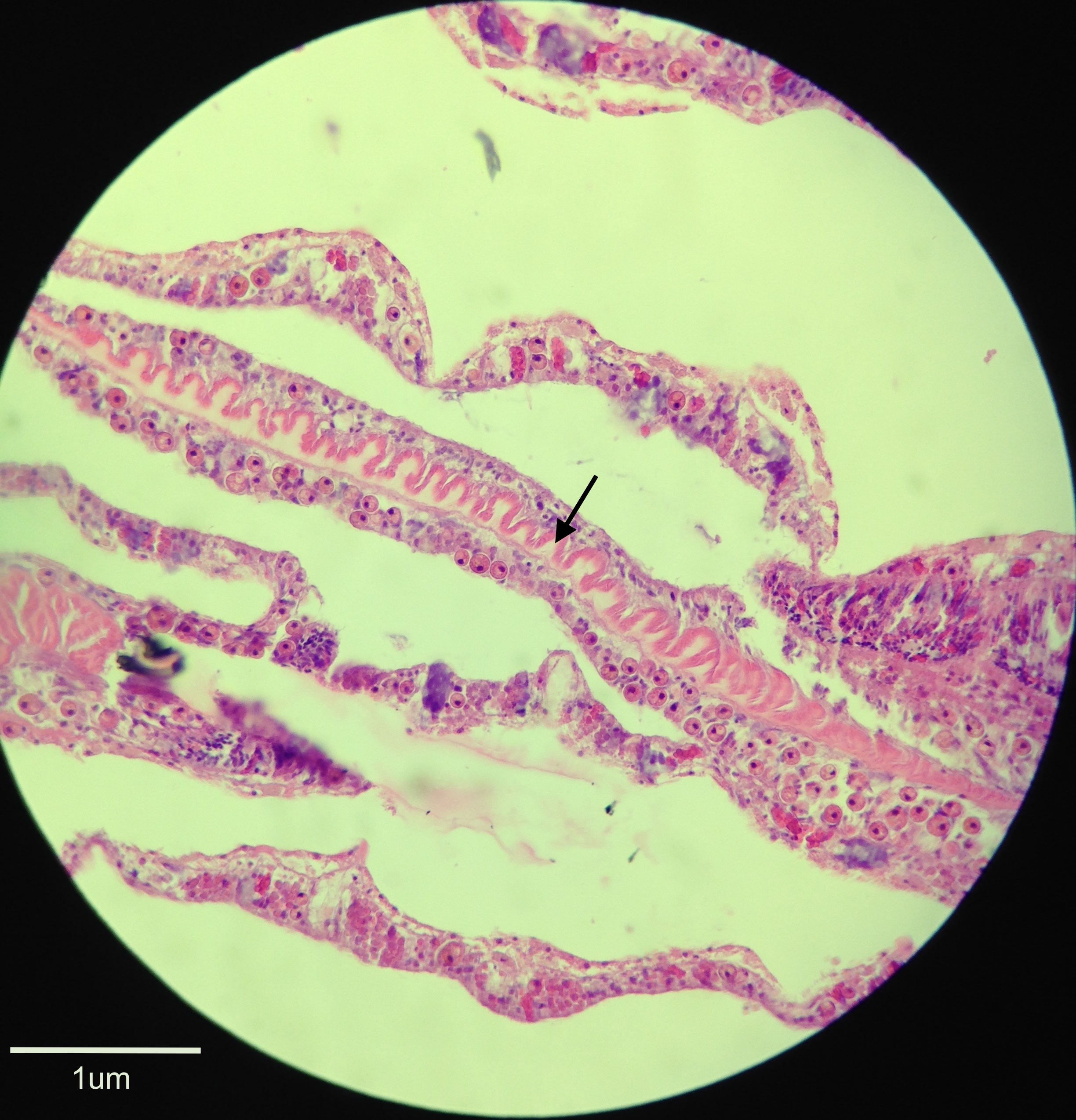
Figure 11. A histological section of a fixed specimen stained with H&E and viewed under 40x magnification. The arrow indicates the mesenterial filament running through the specimen that is stained light pink with a slightly darker pink thickened edge.
|
|
|
Skeletal System | |
When a coral polyp settles on the benthos it begins to secrete a calcium carbonate exoskeleton individually known as a corallite. In terms of the whole colony, this skeleton is collectively called the corallum and the part between each corallite is called the coenosteum (Brusca, Moore & Shusca 2016). The corallite’s outer wall is the theca and it attaches to the substrate with a basal plate, with the whole structure being supported by the columella (Brusca, Moore & Shusca 2016). From this, septa radiate in a predictive pattern definitive of G. fascicularis. Four cycles of septa are typical with the first two usually being exsert and possibly of an irregular shape, however in some deep water colonies the fourth cycle may be absent, or those with large calices may have a partially formed fifth cycle (Veron 1986). For additional support to the polyps, longitudinal and circular muscles combined with the gastrovascular cavity act as a hydrostatic skeleton (Brusca, Moore & Shusca 2016).
|
|
|
Digestive System | |
In the process of intracoelenteric digestion of G. fascicularis, ingested food passes through the pharynx and enters the coelenteron for extracellular digestion (Brusca, Moore & Shusca 2016). Here, digestive enzymes are produced by cells in the gastrodermis that break food down into a soupy mixture consisting of polypeptides, fats, and carbohydrates (Brusca, Moore & Shusca 2016). Circulation of digestive juices in the coelenteron may be aided by ciliary action on the lateral lobes of mesenterial filaments of the gastrovascular cavity (Brusca, Moore & Shusca 2016). These nutrients are then distributed throughout the coelenteron into other parts of the body to be fully digested intracellularly in food vacuoles in nutritive-muscular cells via phagocytosis or pinocytosis into the cell (Brusca, Moore & Shusca 2016). As the gut of the polyp is classified as being blind, all food enters and any undigested wastes exit through the one opening. While G. fascicularis also digests food extracoelenterically via mesentarial filaments, the specific process describing how this occurs has not been recounted in depth and therefore cannot be discussed further.
|
|
|
Circulatory and Excretory Systems | |
Due to their diploblastic nature, there are no true coelomic or hemal cavities within anthozoans. Instead, the coelenteron fulfils the function of circulation and distributes partly digested materials throughout the body, absorbs metabolic wastes contained in the gastrodermis, and eventually expels all wastes through the mouth (Brusca, Moore & Shusca 2016). Due to the polyps lacking a nephridial system, the main nitrogenous excretory product of corals (ammonia) is expelled through the body wall via diffusion where it then dissolves and is carried away by seawater (Ruppert, Fox & Barnes 2004).
|
|
|
Nervous and Sensory Systems | |
Due to the radial symmetry of the animal, the nerve net of cnidarian polyps is diffuse and non-centralised with sense organs being distributed radially (Brusca, Moore & Shusca 2016). Two nerve nets exist with one being bounded by epidermis and mesenchyme and the other gastrodermis and mesenchyme (Brusca, Moore & Shusca 2016). Due to the neurons and synapses of cnidarians being non-polar, impulses generated by stimuli can extend in every direction throughout the nerve net (Brusca, Moore & Shusca 2016). Minuscule hair-like structures located on the body surface, in particular in higher densities on the tentacles and where cnidae are more abundant, may act as mechanoreceptors or chemoreceptors, influencing body movement and movement of tentacles toward prey or predators (Brusca, Moore & Shusca 2016). While polyps display photosensitivity, there is no receptor known to actively facilitate this, and instead it is thought to be associated with the epidermal cell’s translucent surfaces that contain concentrations of neurons within or just below the surface (Brusca, Moore & Shusca 2016).
|
|
|
Muscular System | |
While no true mesoderm exists in cnidarians, longitudinal and circular fibrils that originate from the epithelium and reside in the mesenchyme tend to be loosely termed as muscles (Brusca, Moore & Shusca 2016). Within the tentacles and oral disc, epitheliomuscular cells enable movement, which particularly aids in the capture and transport of prey to the mouth during feeding, or the movement of tentacles in defence (Brusca, Moore & Shusca 2016). While extension of the polyp is mainly achieved through manipulating the amount of water in the hydrostatic skeleton, the column of the polyp can be contracted via longitudinal fibres acting as retractor muscles that are located in bundles lining the mesenteries (Brusca, Moore & Shusca 2016). The gastrodermis produces circular muscles that are found in the tentacles, oral disc and mouth and are especially useful in holding the mouth closed (Brusca, Moore & Shusca 2016). Distension of the polyp body and tentacles is also achieved in combination by the hydrostatic skeleton and circular muscles (Brusca, Moore & Shusca 2016).
|
|
|
Respiration | |
All cnidarians lack specialised organs for gas exchange, thus respiration occurs via diffusion across the internal and external cell surfaces of the polyp’s coelenteron, tentacles and oral disc (Brusca, Moore & Shusca 2016). This supplies the polyp with sufficient gases due to the thin body wall of the polyp (Brusca, Moore & Shusca 2016). As these are sessile creatures, they rely on water currents to bring essential gases to them. Shallow water colonies often have a constant supply of new water brought to them, however deeper water colonies may be in more stagnant water and increase efficiency by creating a water current themselves. This is done by the beating of epidermal cilia, which create a current that draws water over and into the body (Ruppert, Fox & Barnes 2004).
|
|
|
Defences | |
A defining feature of the phylum Cnidaria and a useful defensive mechanism is the cnidae. A fully formed cnidae is termed the more commonly known cnidocyte. Three types of cnidocytes exist within the Cnidarians; true nematocysts, spirocysts, and ptychocysts. Within scleractinian corals and thus G. fascicularis, only nematocysts and spirocysts occur. Nematocysts are defined as having capsules that are double-walled and a barbed tubule to increase the efficiency of penetration and anchorage into the prey (Ruppert, Fox & Barnes 2004). A mixture of phenols and proteins constitute the toxins contained within the capsule, which may be transferred into a wound by being on the tubule surface, or it may be injected through a terminal pore on the thread (Ruppert, Fox & Barnes 2004). In contrast, spirocysts have capsules with only a single wall and their tubules are adhesive rather than barbed and have no apical pore due to the fact that they do not pierce the prey but rather wrap around it (Ruppert, Fox & Barnes 2004). The capsules in this case also contain mucoprotein or glycoprotein rather than toxins (Ruppert, Fox & Barnes 2004). A nematocyst or spirocysts’ release may be triggered by mechanical and chemical stimuli, resulting in the tripartite apical flap covering the coiled barb opening, releasing the cnidae into the prey (Ruppert, Fox & Barnes 2004). Figure 12 depicts these cnidocytes in the coelenteron and tentacle where they are most abundant. Whether these are nematocysts or spirocysts however, could not be determined.
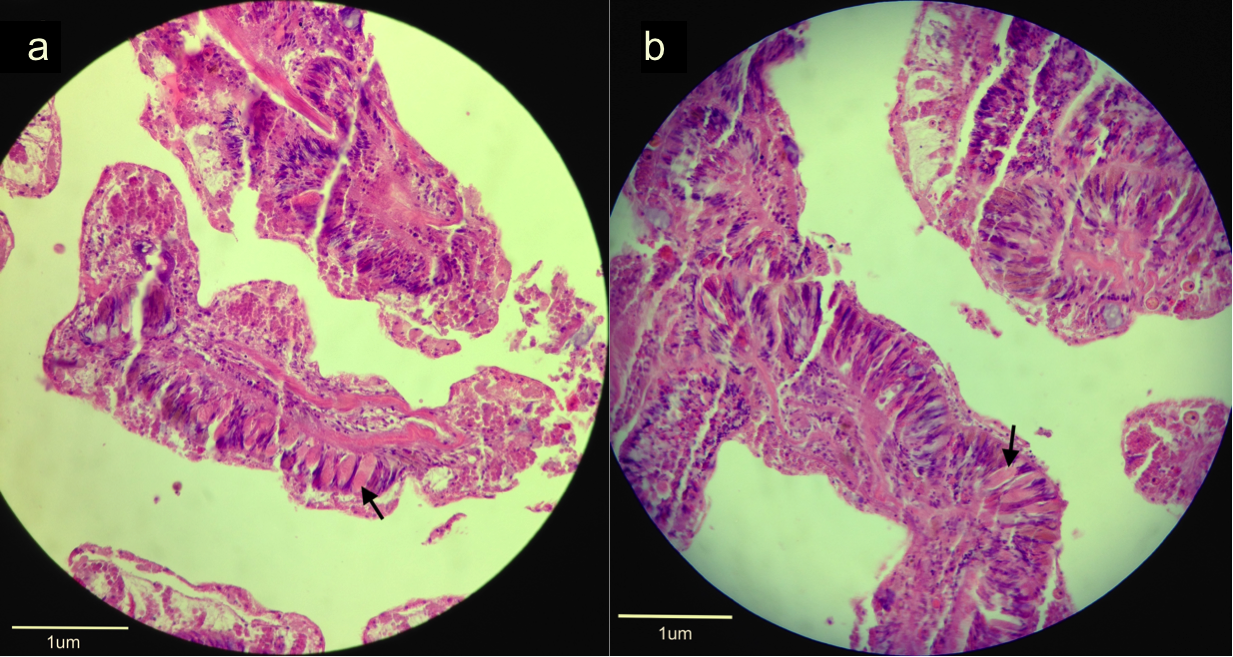
Figure 12. Histological sections of a fixed specimen stained with H&E and viewed under 40x magnification. Arrows indicate the cnidocytes (stained light pink) within (a) the coelenteron and (b) a tentacle.
Corals can deal with interspecific competition for space through use of sweeper tentacles by discharging nematocysts at their opponent, by expelling mesenterial filaments for extracoelenteric digestion, or through overgrowth (Hidaka 1985). When intraspecific competition occurs between two colonies of G. fascicularis, sweeper tentacles have been the main described mechanism of defence, however interestingly are only utilised when the competing colony is of a different colour morph (Hidaka 1985; Hidaka & Yamazoto 1984). The composition of nematocysts of the sweeper tentacles is also different to those of regular tentacles, and it is thought that sweeper tentacles form from regular tentacles, due to the presence of tentacles at intermediate states in terms of their outward appearance and nematocyst composition (Hidaka & Yamazoto 1984).
|
|
|
Biofluorescence | |
The ability to fluoresce has been observed in many different marine invertebrates with corals being considered one of the most fluorescent animals in the sea. The significant applications of fluorescence however, in particular in molecular and cellular biology, have only recently become recognised. One of the most well known fluorescent proteins, the green fluorescent protein (GFP), was first discovered in the jellyfish Aequorea victoria in 1961 and has since been used for a myriad of techniques such as molecular markers, fusion tags, transcriptional reporters and biosensors (Ben-Zvi, Eyal & Loya 2015; Karasawa et al. 2003). These pigments have the ability to autocatalytically produce a specialised molecule called a chromophore that causes fluorescence by absorbing light that is of a short wavelength and then re-emitting it at a longer wavelength thus giving off the green colour (Ben-Zvi, Eyal & Loya 2015; Vogt et al. 2008). The specific function of GFP in coral has been widely discussed with possible roles including antioxidant function (Bou Abdallah et al. 2006), photoprotection (Salih et al. 2000), attraction of zooxanthellae (Hollingsworth et al. 2004), and photosynthesis facilitation (Dove et al. 2008).
In G. fascicularis, GFP has been identified and exists in different amounts depending on the colour morph of the coral colony (Nakaema & Hidaka 2015). Figure 13 displays the biofluorescence displayed in one of the specimens when viewed under blue light with a wavelength of 475nm. Within this species, GFP was proposed to have the function of being photoprotective in the study conducted by Ben-Zvi et al. (2015), however the study by Nakaema and Hidaka (2015) concluded that the GFP of the corals in their study did not have a role of photoprotection. Thus further investigation is still needed in order to determine what the specific role may be of GFP in G. fascicularis.
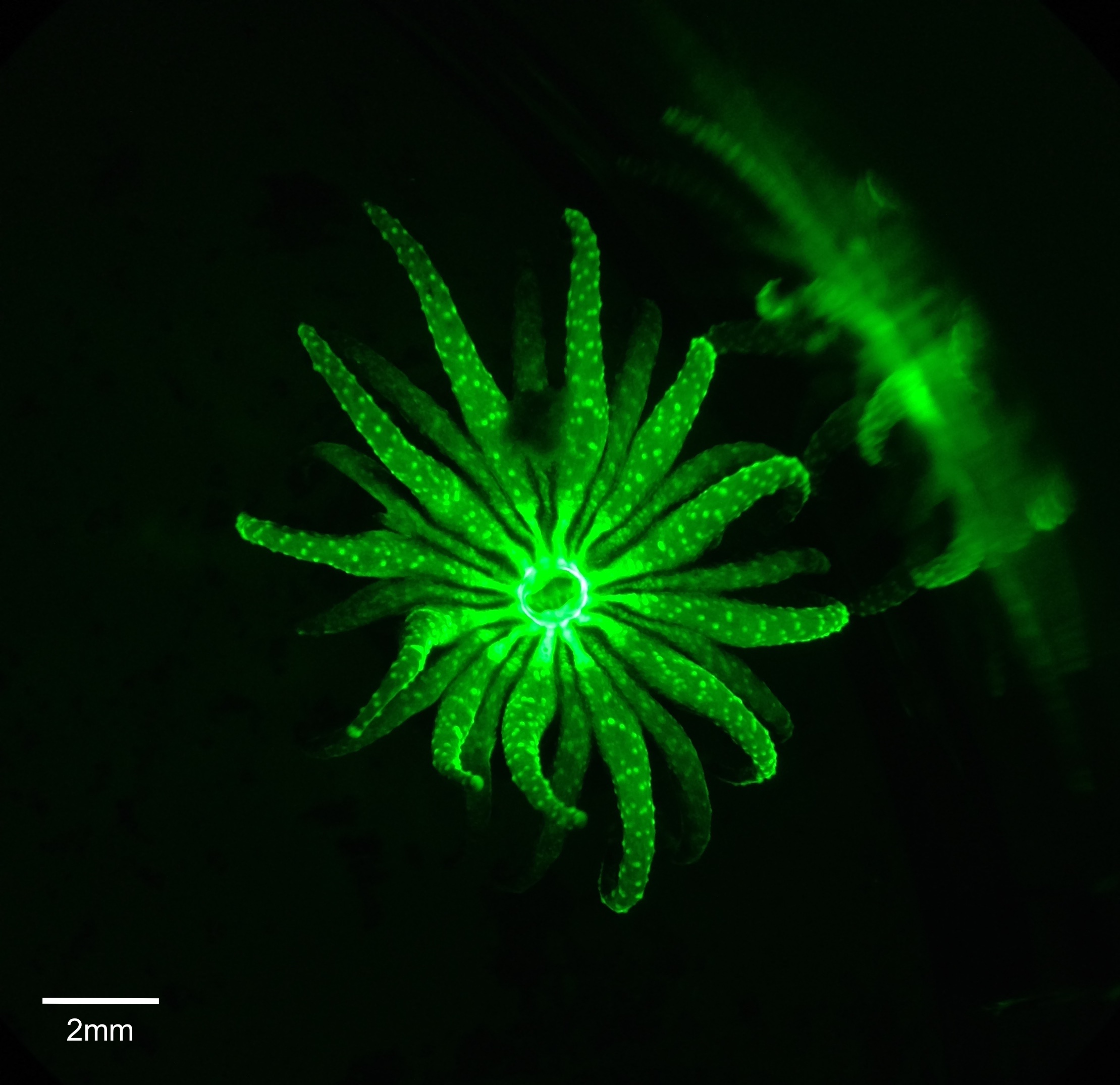
Figure 13. Live polyp viewed under blue light to display biofluorescence caused by a green fluorescent protein.
|
|
|
|
Biogeographic Distribution | |
G. fascicularis occurs across a wide variety of latitudes where optimal conditions for reef-building corals such as oligotrophic, warm tropical and subtropical waters exist. This species is distributed across the Indo-Pacific ranging from the Red Sea to as far east as Samoa and Fiji and including the Arabian Gulf, east Africa, South East Asia and Australia in between (Fig. 14) (Veron & Pichon 1979; Veron 2000). Extensive studies have occurred on samples located on the Great Barrier Reef, Australia (Harrison 1989; Stafford-Smith 1993), Wakatobi Marine National Park in Indonesia (Crabbe & Smith 2006), Okinawa, Japan (Hidaka & Yamazoto 1984; Karasawa et al. 2003; Abe et al. 2008; Nakajima et al. 2015), Taiwan (Keshavmurthy et al. 2012), the Gulf of Aqaba, Jordan (Al-Horani et al. 2005), the South China Sea (Huang et al. 2011; Chen et al. 2013), Malaysia (Hii, Soo & Liew 2009), and Papua New Guinea (Smith et al. 2016). The genetic connectivity of G. fascicularis has not been extensively detailed in the literature and thus could not be commented on in sufficient detail.
Figure 15. Global range of G. fascicularis (adapted from Hoeksema, Rogers & Quibilan 2014).
|
|
|
Evolution and Systematics | |
Phylum Cnidaria
The evolution and phylogeny of Cnidarians starts from when the first fossil evidence of these metazoans arose in the Ediacaran period (Brusca, Moore & Shusca 2016). The Cnidarian body plan has remained largely unchanged since the Cambrian period and possibly evolved from either a diploblastic planuloid or a triploblastic acoelomate organism (Brusca, Moore & Shusca 2016). Phylogenetic, morphological and paleontological data has been utilised to determine the phylogenetic history and evolutionary relationships of the taxa within Cnidaria. The four classes that exist are under ongoing debate regarding their position, with two main views giving two possibilities. One view is that Cnidaria are a monophyletic clade based on the medusoid hypothesis, which states that a medusoid body form representing the sexual stage was ancestral thus giving the tree in Figure 15a (Brusca, Moore & Shusca 2016; Bridge et al. 1992). In contrast another view, the polypoid hypothesis, which is more supported, states that the polyp form of anthozoa evolved first, and the remaining three clades diverged from this with the medusoid form arising later (Figure 15b) (Brusca, Moore & Shusca 2016).
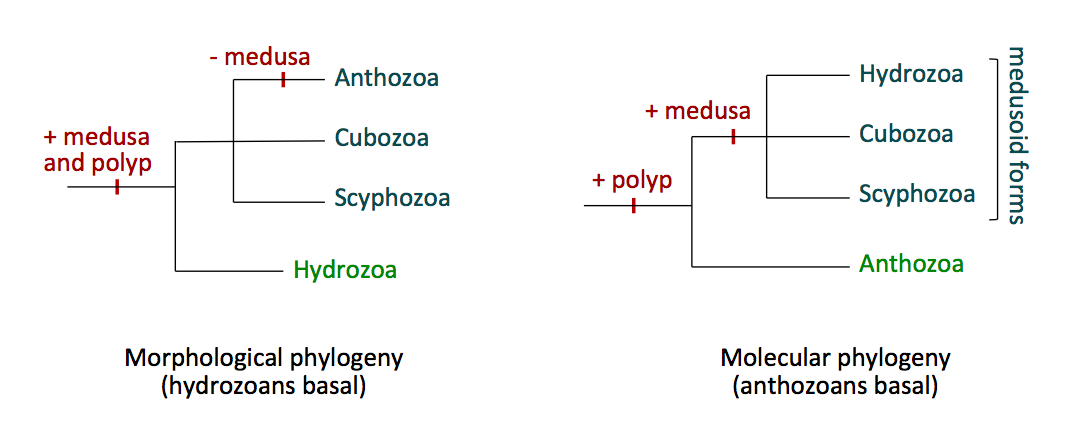
Figure 15. Two possible configurations of the classes within the phylum Cnidaria; (a) the phylogenetic tree based on the medusoid hypothesis and (b) the phylogenetic tree based on the polyp hypothesis. Sourced from the 'Phylum Cnidaria' lecture notes from the course BIOL3211, University of Queensland (2017).
Class Anthozoa
Anthozoans have previously been thought of as a monophyletic group based on morphological synapomorphies. These are that the polyps are bilaterally symmetrical, the coelenteron has an actinopharynx, mesenterial filaments and syphonoglyph, nematocysts do not have an operculum, the cnidae have tripartite flaps, and cnidocytes have special ciliary cones associated with them (Marques & Collins 2004; Brusca, Moore & Shusca 2016). Based on phylogenetic analyses, however, the Octocorallia may have a closer affiliation to the Medusozoa than the Hexacorallia, thus resulting in the class being paraphyletic (Kayal & Lavrov 2008).
Order Scleractinia
Scleractinia have a fossil record spanning back 250 million years, however weak paleontological evidence and variation in morphology make defining characters of the order hard to distinguish. The 24 families included in the order do however share the feature of having an aragonite calcium carbonate skeleton with insertion of septa occurring cyclically (Romano & Cairns 2000). Genetic analyses however show that the skeleton has evolved multiple times within the order and this results in it being polyphyletic rather than monophyletic as the latter morphologies imply (Romano & Cairns 2000). Within this order, two distinct clades exist; “robust” which incorporates species with skeletons that are compact and heavily calcified, and “complex” that have more skeletons of higher complexity that are lighter and more porous (Brusca, Moore & Shusca 2016).
Family Oculinidae
This family is classed as being polyphyletic as multiple genus fall into different categories regarding the complex or robust clade of Scleractinia (Lin et al. 2012; Kitahara et al. 2010). Defining morphological features include vesicular coenenchyma, highly exsert septa and ramose or plocoid growth forms of zooxanthellate corals that are reef-builders (Eguchi 1942; Romano & Cairns 2000). Specific phylogenetic analyses for the classification of the family Oculinidae were unable to be sourced.
Genus Galaxea
The genus Galaxea first appeared in the fossil record possibly during the Eocene period from fossils in the Indian Ocean, however a more supported fossil appears in the Oligocene from deposits of the Caribbean and Tethys (Veron 2000). There are incongruences in the literature as to which family this genus belongs to, with some papers suggesting it be moved to the Euphylliidae family, however due to the fact that many recent papers list it in the Oculinidae this was the classification used for this web page. The genus contains seven species (Nakajima et al. 2015).
Species fascicularis
In a study conducted by Abe et al. (2008) colonies of G. fascicularis in Okinawa, Japan could be divided into two genetically distinct groups based on the morphology of their nematocysts that differ in their mt genotype and MpM morphology. It was found that a nearly impermeable reproductive barrier impeded breeding between these two sympatric groups as evidenced by only two individuals displaying a combination of the alleles that would be expected in hybrids (Abe et al. 2008). This may provide evidence of cryptic or sibling species existing within this taxon. Nakajima et al. (2015) also suggest that novel clades or species may be present based on the differential classification into hard and soft groups. There is also debate over whether this species is classified as complex or robust, with its placement in the family Oculinidae resulting in it being complex, while those who suggest it be placed in the family Euphyliidae results in it being robust (Okubo et al. 2013; Okubo 2016).
|
|
|
Conservation and Threats | |
Coral reefs are some of the most biodiverse ecosystems on the planet, supporting a range of endemic organisms as well as the ways of life of many people from all over the world. Despite this however, there is an increasing number of direct and indirect threats to coral reefs, threatening to deteriorate them to a point beyond return. Due to its relatively large distribution and population connectivity, G. fascicularis is not as at risk as other coral species, however there are still many possible pressures threatening the health of this species and thus scientific study is still needed to ensure the continuation of this species and coral reef productivity as a whole.
Rising temperatures are generally considered to pose a great threat to corals as they can result in bleaching and increased susceptibility to disease. Bleaching occurs when the corals are exposed to temperatures beyond those they are capable of tolerating, resulting in expulsion of their zooxanthellae due to thermal stress (Wilkinson 2004). This is problematic for the corals as they obtain essential nutrients through their symbiont’s photosynthesis. While the corals may be able to reacquire zooxanthellae once temperatures decrease, prolonged stress will prevent this from being possible and result in algae overgrowing the coral, eventually starving it of nutrients and killing it (Wilkinson 2004). It has also been shown in a study on G. fascicularis that higher temperatures decreased the respiration rates of the coral, thus limiting the ability of zooxanthellae to photosynthesise as they use the CO2 produced by the corals (Agostini et al. 2013). Throughout history many coral bleaching events have occurred and appear to be on the rise with increasing amounts of reefs becoming bleached each time. Some Symbiodinium clades may provide an advantage and it is suggested that corals may adapt to increased temperature by switching their affiliations with the zooxanthellae to these clades (Wilkinson 2004).
The stress of increased temperatures to corals also causes them to become more susceptible to diseases, the number of which has drastically increased in recent years along with the number of coral species affected (Porter et al. 2001). Anthropogenic processes such as pollution and overfishing of organisms that control diseases, as well as increasing sea surface temperatures are thought to be some of the leading causes of increased diseases (Wilkinson 2004). Infections have the potential to critically deteriorate critical reef species, leading to irreversible damage to the structure of reefs. For this reason, further research must be conducted to evaluate the threat individual species may incur.
With increased production and release of CO2 into the atmosphere, there is increased absorption of CO2 into the ocean, resulting in a drop in pH making the ocean a more acidic environment (Agostini et al. 2013). With this shift in carbon balance comes a lower aragonite saturation state that is thought to decrease the calcification rates of corals building their skeletons (Agostini et al. 2013). The pH within the subcalicoblast space of the polyp where calcification occurs must be maintained at an optimal level in order for calcium carbonate to be precipitated (Agostini et al. 2013). Thus a decrease of pH in the external environment also results in a decrease of pH in the internal environment of the polyp, diminishing the rate of calcification produced by the coral (Agostini et al. 2013). It was also found that the effect of increased acidity differed based on whether it was night time or daytime. During the day, the photosynthesising of the zooxanthellae seemed to mitigate the effect by consuming CO2 resulting in little change to the pH of the microenvironment surrounding the corals (Agostini et al. 2013). At night however this photosynthesis does not occur and thus the corals are affected more by the increased pH. Despite the vast amount of science supporting this view of the effect ocean acidification has on corals, a recent paper by Von Euw et al. (2017) claims that calcification is a completely biological process that is regulated by the animal and not affected by the abiotic features of the external environment. With further research, the fate of coral calcification with increasingly acidic oceans will become more clear.
Crown of thorns starfish (COTS) outbreaks have also increased, most likely as a result of anthropogenic effects such as removal of their predators (Wilkinson 2004). COTS cause major damage to coral reefs as they consume coral, with Dumas et al. (2016) comparing their ability to degrade reef ecosystems to that of cyclones. When outbreaks occur, vast quantities of a reef can be destroyed such that there are major declines in biodiversity, and structural complexity and overall biomass productivity decreases (Dumas et al. 2016). The trophic cascade caused by COTS is also of concern to the livelihoods of many people, especially those of small island communities where the reef plays a significant role in their life.
G. fascicularis is a highly sought-after coral for the aquarium trade and is thus at risk from over-harvesting. While quotas may be implemented to limit the number of pieces exported, this is often ignored with far more being collected. Primary literature on the commercial harvest of this species could not be found, however the IUCN state that the largest exporter is Indonesia with the year 2005 seeing a global total of 20686 pieces (live and raw) exported for the aquarium trade (Hoeksema, Rogers & Quibilan 2014).
Based on the IUCN classification, G. fascicularis is currently in the ‘Near threatened’ category, thus further exacerbation of these threats may result in higher risks to the species and consequently conservation is a useful tool for protecting them, especially when implemented on coral reefs as a whole. At present, Marine Protected Areas (MPAs) are one of the most common methods used in coral conservation, however there are difficulties associated with them, as biodiversity hotspots and sites of high endemism rarely overlap (Hughes, Bellwood & Connolly 2002). Regardless of this however, they provide protection to species at risk by reducing the effects of fishing such as trophic cascades that result when too many individuals of a species are removed and habitat degradation by trawlers, increase population connectivity through interconnected protection of larvae, minimize the loss of biodiversity, and prevent the harvesting of threatened species by implementing no-take zones, quotas and size limits (Wilkinson 2004). For continued and future conservation of this species, further research into its biology and ecology, taxonomy, population connectivity and size, threats and resilience to these, disease and parasite management, and MPA success or expansion need to be conducted.
|
|
|
References | |
Abe, M., Watanabe, T., Suzuki, Y. & Hidaka, M. 2008. Genetic and morphological differentiation in the hermatypic coral Galaxea fascicularis in Okinawa, Japan. Plankton Benthos Research. 3, 174-179.
Agostini, S., Fujimura, H., Higuchi, T., Yuyama, I., Casareto, B.E., Suzuki, Y. & Nakano, Y. 2013. The effects of thermal and high-CO2 stresses on the metabolism and surrounding microenvironment of the coral Galaxea fascicularis. Comptes Rendus Biologies. 336, 384-391.
Babcock, R.C. & Heyward, A.J. 1986. Larval development
of certain gamete-spawning scleractinian corals. Coral Reefs. 5, 111-116.
Ben-Zvi, O., Eyal, G. & Loya, Y. 2015. Light-dependent fluorescence in the coral Galaxea fascicularis. Hydrobiologia. 759, 15–26.
Bou-Abdallah, F., Chasteen, N.D. & Lesser, M.P. 2006. Quenching of superoxide radicals by green fluorescent protein. Biochimica et Biophysica Acta. 1760, 1690–1695.
Bridge, D., Cunningham, C.W., Schierwater, B., Desalle, R. & Buss, L.W. 1992. Class-level relationships in the phylum Cnidaria: Evidence from mitochondrial genome structure. Proceedings of the National Academy of Sciences USA. 89, 8750-8753.
Brusca, R.C., Moore, W. & Shusca, S.M. 2016. Invertebrates, 3 ed. Sinauer Associates, Sunderland, Massachusetts, USA.
Chen, D., Wang, D., Zhu, J., Li, Y., Wu, X. & Wang, Y. 2013. Identification and characterization of microsatellite markers for scleractinian coral Galaxea fascicularis and its symbiotic zooxanthellae. Conservation Genetics Resources. 5, 741–743.
Crabbe, M.J.C. & Smith, D.J. 2006. Modelling variations in corallite morphology of coral colonies with depth and light on coastal fringing reefs in the Wakatobi Marine National Park (S.E. Sulawesi, Indonesia). Computational Biology and Chemistry. 30, 155-159.
Dong, Z., Huang, H., Huang, L. & Li, Y. 2009. Diversity of symbiotic algae of the genus Symbiodinium in scleractinian corals of the Xisha Islands in the South China Sea. Journal of Systematics and Evolution. 47, 321–326.
Dove, S. G., Lovell, C. Fine, M., Deckenback, J., Hoegh-Guldberg, O., Iglesias-Prieto, R. & Anthony, K. 2008. Host pigments: potential facilitators of photosynthesis in coral symbioses. Plant, cell & environment. 31, 1523–1533.
Dumas, P., Moutardier, G., Ham, J., Kaku, R., Gereva, S., Lefèvre, J. & Adjeroud, M. 2016. Timing within the reproduction cycle modulates the efficiency of village-based crown-of-thorns starfish removal. Biological Conservation. 204, 237-246.
Eguchi, M. 1942. Recent and fossil corals of the family Oculinidae from Japan. Transaction of the Palaeontological Society of Japan. 147,139-147.
Grottoli, A.G., Rodrigues, L.J. & Palardy, J.E. 2006. Heterotrophic plasticity and resilience in bleached corals. Nature. 440, 1186-1189.
Harrison, P.L. 1989, ‘Pseudo-gynodioecy: an unusual breeding system in the scleractinian coral Galaxea fascicularis’, Proceedings of the Sixth International Coral Reef Symposium. 2, 699-705.
Hidaka, M. 1985. Nematocyst Discharge, Histoincompatibility, and the Formation of Sweeper Tentacles in the Coral Galaxea fascicularis. Biological Bulletin. 168, 350-358.
Hidaka, M. & Yamazato, Y. 1984. Intraspecific Interactions in a Scleractinian Coral,
Galaxea fascicularis: Induced Formation of Sweeper Tentacles. Coral Reefs. 3, 77-85.
Hii, Y., Soo, C. & Liew, H. 2009. Feeding of scleractinian coral, Galaxea fascicularis, on Artemia salina nauplii in captivity. Aquaculture International. 17, 363–376.
Hoeksema, B.W., Rogers, A. & Quibilan, M.C. 2014. Galaxea fascicularis. The IUCN Red List of Threatened Species 2014. Viewed 26 May 2017, <http://www.iucnredlist.org/details/133662/0>.
Hollingsworth, L., Krupp, D., Lewis, T., Leong, J. 2004. Early onset and expression of uorescent protein in the larvae of the mashroom coral Fungia scutaria Lamarck 1801. Proceedings of the Tenth International Coral Reef Symposium. 99-105.
Huang, H., Dong, Z., Huang, L., Yang. J., Di, B., Li, Y., Zhou, G. & Zhang, C. 2011. Latitudinal variation in algal symbionts within the scleractinian coral Galaxea fascicularis in the South China Sea. Marine Biology Research. 7, 208-211.
Hughes, T.P., Bellwood, D.R. & Connolly, S.R. 2002. Biodiversity hotspots, centres of endemicity, and the conservation of coral reefs. Ecology Letters. 5, 775–784.
Junjie, R.K., Browne, N.K., Erftemeijer, P.L.A. & Todd, P.A. 2014. Impacts of Sediments on Coral Energetics: Partitioning the Effects of Turbidity and Settling Particles. PLoS ONE. 9, 1-11.
Karasawa, S., Araki, T., Yamamoto-Hino, M. & Miyawaki, A. 2003. A Green-emitting Fluorescent Protein fromGalaxeidae Coral and Its Monomeric Version for Use in Fluorescent Labeling. The Journal Of Biological Chemistry. 278, 34167-34171.
Kayal, E. & Lavrov, D.V. 2008. The mitochondrial genome of Hydra oligactis (Cnidaria, Hydrozoa) sheds new light on animal mtDNA evolution and cnidarian phylogeny. Gene, 410, 177–186.
Keshavmurthy, S., Hsu, C., Kuo, C., Denis, V., Leung, J.K., Fontana, S., Hsieh, H.J., Tsai, W., Su, W. & Chen, C.A. 2012. Larval Development of Fertilized “Pseudo-Gynodioecious” Eggs Suggests a Sexual Pattern of Gynodioecy in Galaxea fascicularis (Scleractinia: Euphyllidae). Zoological Studies. 51, 143-149.
Kitahara, M.V., Cairns, S.D., Stolarski, J., Blair, D., Miller, D.J. 2010. A Comprehensive Phylogenetic Analysis of the Scleractinia (Cnidaria, Anthozoa) Based on Mitochondrial CO1 Sequence Data. PLoS ONE. 5, 1-9.
Lin, M., Kitahara, M.V., Tachikawa, H., Fukami, H., Miller, D.J. & Chen, C.A. 2012. Novel organization of the mitochondrial genome in the deep-sea coral, Madrepora oculata (Hexacorallia, Scleractinia, Oculinidae) and its taxonomic implications. Molecular Phylogenetics and Evolution. 65, 323–328.
Nakaema, S. & Hidaka, M. 2015. Fluorescent protein content and stress tolerance of two color morphs of the coral Galaxea fascicularis. Galaxea, Journal of Coral Reef Studies. 17, 1-11.
Nakajima, Y., Zayasu, Y., Shinzato, C., Satoh, N. & Mitarai, S. 2016. Genetic differentiation and connectivity of morphological types of the broadcast-spawning coral Galaxea fascicularis in the Nansei Islands, Japan. Ecology and Evolution. 6, 1457– 1469.
Nakajima, Y., Shinzato, C., Satoh, N. & Mitarai, S. 2015. Novel Polymorphic Microsatellite Markers Reveal Genetic Differentiation between Two Sympatric Types of Galaxea fascicularis. PLoS ONE. 10, 1-12.
Nakano, Y. 2009. Intratentacular budding in Galaxea fascicularis. Galaxea, Journal of Coral Reef Studies. 11, 37.
Okubo, N. 2016. Restructuring the Traditional Suborders in the Order Scleractinia
Based on Embryogenetic Morphological Characteristics. Zoological Science 33, 116–123.
Okubo, N., Mezaki, T., Nozawa, Y., Nakano, Y., Lien, Y., Fukami, H., Hayward, D.C. & Ball, E.E. 2013. Comparative Embryology of Eleven Species of Stony Corals (Scleractinia). PLoS ONE. 8, 1-22.
Porter, J.W., Dustan, P., Jaap, W.C., Patterson, K.L., Kosmynin, V., Meier, O.W., Patterson, M.E. & Parsons, M. 2001. Patterns of spread of coral disease in the Florida Keys. Hydrobiologia. 460, 1–24.
Rieger, R.M. 1994. The Biphasic Life Cycle: A Central Theme of Metazoan Evolution. American Zoologist. 34, 484-491.
Romano, S.L. & Cairns, S.D. 2000. Molecular phylogenetic hypotheses for the evolution of scleractinian corals. Bulletin Of Marine Science. 67, 1043–1068.
Ruppert, E.E., Fox, R.S. & Barnes, R.D. 2004. Invertebrate Zoology: A Functional Evolutionary Approach, 7 ed, Brookes/Cole, USA.
Salih, A., Larkum, A., Cox, G., Kuhl, M. & Hoegh-Guldberg, O. 2000. Fluorescent pigments in corals are photoprotective. Nature. 408, 850–853.
Scott. P.J.B. 1984. The Corals of Hong Kong. Hong Kong University Press, Hong Kong.
Smith, J.N., Strahl, J., Noonan, S.H.C., Schmidt, G.M., Richter, C. & Fabricius, K.E. 2016. Reduced heterotrophy in the stony coral Galaxea fascicularis after life- long exposure to elevated carbon dioxide. Scientific Reports. 6, 1-10.
Veron, J.E.N. 2000. Corals of the world. Australian Institute of Marine Science, Townsville, Australia.
Veron, J.E.N. 1986. Corals of Australia and the Indo-Pacific. Angus & Robertson, North Ryde, NSW, Australia.
Veron, J.E.N. & Pichon, M. 1979. Scleractinia of Eastern Australia: Part III. Australian Institute of Marine Science, Townsville, Australia.
Vogt, A., D’Angelo, C., Oswald,F., Denzel, A., Mazel, C.H., Matz, M.V., Ivanchenko, S., Nienhaus, G.U. & Wiedenmann, J. 2008. A Green Fluorescent Protein with Photoswitchable Emission from the Deep Sea. PLoS ONE. 3, 1-8.
Von Euw, S., Zhang, Q., Manichev, V., Murali, N., Gross, J., Feldman, L.C., Gustafsson, T., Flach, C., Mendelsohn, R. & Falkowski, P.G. 2017. Biological control of aragonite formation in stony corals. Science. 356, 933-938.
Wijgerde, T., Schots, P., Van Onselen, E., Janse, M., Karruppannan, E., Verreth, J.A.J. & Osinga, R. 2013. Epizoic acoelomorph flatworms impair zooplankton feeding by the scleractinian coral Galaxea fascicularis. Biology Open. 2, 10-17.
Wijgerde, T., Diantari, R., Lewaru, M.W., Verreth, J.A.J. & Osinga, R. 2011. Extracoelenteric zooplankton feeding is a key mechanism of nutrient acquisition for the scleractinian coral Galaxea fascicularis. The Journal of Experimental Biology. 214, 3351-3357.
Wilkinson, C. 2004. Status of the Coral Reefs of the World: 2004. Volume 1. Australian Institute of Marine Science, Townsville, Australia.
|
|
|
|
|